Thank you for visiting nature.com. You are using a browser version with limited support for CSS. To obtain the best experience, we recommend you use a more up to date browser (or turn off compatibility mode in Internet Explorer). In the meantime, to ensure continued support, we are displaying the site without styles and JavaScript.
Scientific Reports volume 13, Article number: 15607 (2023 ) Cite this article Red Algae
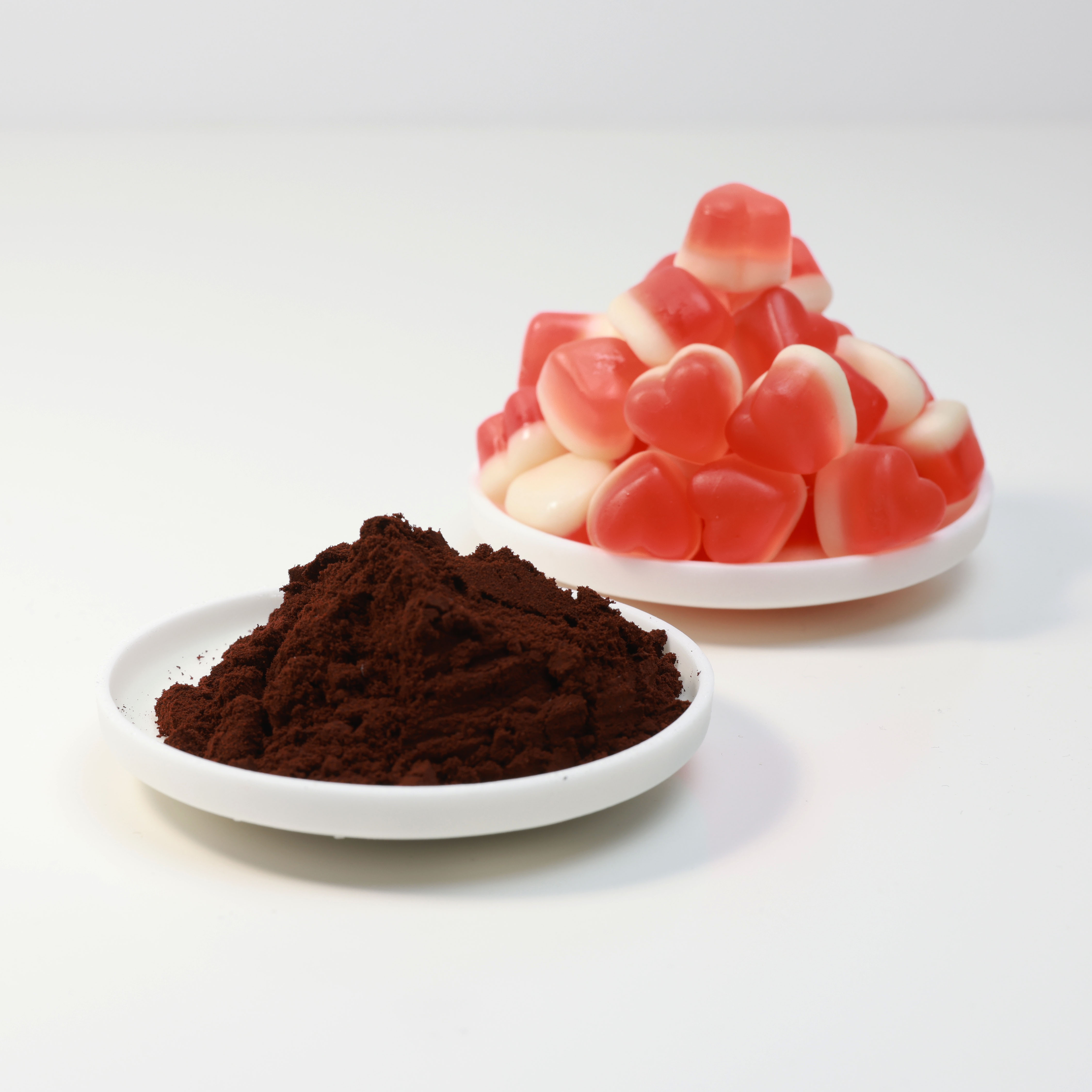
Docosahexaenoic acid (DHA) and selenium (Se) are nutrients that confer several health benefits to both humans and animals. Widespread use of DHA in milk powder and health products requires large-scale mass production via Schizochytrium sp., while Se intended for human consumption is produced as organic Se via yeast. However, producing these nutrients on an industrial scale is constrained by various factors. We found that supplementing Schizochytrium sp. with Na2SeO3 (0.5 mg/L) improves its biomass and DHA production and also provides organic Se. De novo assembled transcriptome and biochemical indicators showed that Na2SeO3 promotes forming acetyl coenzyme A and L-cysteine via the glycerol kinase and cysteine synthase pathways, promoting DHA synthesis through the polyketide synthase pathway. However, high doses of Na2SeO3 (5 mg/L) limited the biomass of Schizochytrium sp. and DHA content. This study provided a theoretical basis for the simultaneous production of organic Se and DHA via Schizochytrium sp.
Schizochytrium spp. are unicellular, spherical marine fungi considered important biological resources. These species are commonly used to industrially produce docosahexaenoic acid (DHA) because of their stable growth, rapid division, and high content of DHA. DHA is an important long-chain polyunsaturated fatty acid found in the brain, liver, nerve tissues, and other organs of mammals. DHA promotes human brain development during infancy and improves intelligence1. Birch et al.2 found that adding DHA to infant formula can improve the future visual development of term infants. DHA also affects amyloid proteins and the blood–brain barrier carrier protein, APOɛ-4, alleviating the high incidence of Alzheimer’s disease in the elderly3,4. DHA reduces blood lipid levels and lowers the risk of cardiovascular diseases5. Furthermore, DHA plays a neuroprotective role by affecting the cell membrane thickness via acyl groups, the formation of which involves phospholipids, cell receptors, pathways, and peripheral proteins. Therefore, DHA may be considered indispensable to the human body. The factors and mechanisms that influence DHA production by Schizochytrium spp. have been discussed previously. The fatty acid synthase (FAS) pathway is not a major pathway involved in DHA synthesis in Schizochytrium spp; the synthesis of DHA in Schizochytrium sp. is catalyzed via the polyketide synthase (PKS) pathway rather than relying on carbon-chain-elongation reactions catalyzed by dehydrogenation or desaturation enzymes6. This finding provides a new method for studying the mechanisms underlying DHA production in Schizochytrium spp. However, the specific synthetic mechanisms and factors influencing DHA synthesis remain unclear.
Selenium (Se) is an important trace element in the human body, and its deficiency may cause many diseases. Due to the scarcity of Se on land, it must be supplied via food to meet worldwide demand7. Although organic Se produced by yeast is a high-quality source of Se that the human body can use, its use is limited by high production costs8. The utilization of Se by microorganisms is crucial for circulating and transforming Se forms in nature, indicating that Se plays a vital role in microbial metabolism9. Although excessive Se inhibits the growth and metabolism of microorganisms, some microorganisms can tolerate moderate amounts of Se10. In theory, the ability of Schizochytrium spp. to simultaneously produce DHA and organic Se may enable it to be used in a low-cost, high-value-added production system. Because DHA is present in oil, and organic Se is present in proteins, the two can be separated using simple processing technology. Although only a few studies have researched this production mode, it may allow full use of the resources and reduce costs, qualifying it as a potentially widely used method.
Schizochytrium sp. 20,888, available from the US Strain Collection (ATCC), and stored in ATCC using + 790 medium at − 80 °C was used; the culture medium (basic fermentation medium) involved Dextrose 90g/l (Qiqihar Longjiang Fufeng Biotechnology Co., Ltd.). Yeast powder 12g/l (Beijing Hongrun Baoshun Technology Co., Ltd.), Milk peptone 10g/l (Beijing Hongrun Baoshun Technology Co., Ltd.), Sea salt 20g/l (Jinan Zichen Chemical Co., Ltd.) were used. Detection kits for malondialdehyde (MDA), glutathione peroxidase (GSH-Px), acetyl coenzyme A (A-CoA), and reactive oxygen species (ROS) were purchased from Nanjing Jiancheng Biotechnology Co., Ltd. De novo transcriptome assembly was performed by BGI Genomics Co., Ltd.
The sample was randomly divided into five groups: C, T1, T2, T3, and T4, using a single-factor design. Group C (the control group) was cultured in a basic fermentation medium, while groups T1, T2, T3, and T4 were treated with low (0.1 mg/L), medium (0.5 mg/L), high (1 mg/L), and ultra-high (5 mg/L) doses of sodium selenite (Na2SeO3) based on the C group, respectively, with three parallel replications being performed in each group. After 72 h of fermentation, the liquid was dried via centrifugation (4400 × g) and stored in liquid nitrogen for the de novo transcriptome assembly and detection of other indicators. The indicators targeted included the biomass, DHA content, and Se content, and the levels of MDA, GSH-Px, A-COA, and ROS.
Briefly, the procedure involved the following: (1) Total RNA was processed via mRNA enrichment or rRNA removal; enrichment of mRNA where mRNA with PolyA tail was enriched using magnetic beads with oligodt; (2) Removal of rRNA, where rRNA was hybridized with a DNA probe, DNA/RNA hybridization chain was selectively digested using RNaseH, and the DNA probe was digested with DNaseI, to obtain the required RNA after purification; (3) the obtained RNA was fragmented using interrupt buffer and reverse transcribed with random N6 primers, following which the cDNA double strand was used to form double stranded DNA; (4) The end of the synthesized double stranded DNA was flattened and phosphorylated at the 5’ end, whence the 3’ end formed a sticky end protruding an “a”, which was connected to a bubbly joint with a protruding “T” at the 3’ end; (5) The linked products were amplified via PCR using specific primers; (6) The PCR product was thermally denatured into a single strand, and then a bridge primer was used to cyclize the single strand DNA to obtain a single strand circular DNA library; and (7) computer sequencing was performed.
First, low-quality reads with joint contamination and a high content of unknown base N were filtered out. Filtered data were considered clean reads. Clean reads were assembled to obtain UniGene, using which functional annotation and SSR detection were performed. Next, we calculated the expression level of each sample based on UniGene, detected differentially expressed genes (DEGs) between the different samples, annotated the genetic function of the sequencing results with reference to the seven databases, and performed an in-depth cluster analysis and a functional enrichment analysis of the DEGs.
The RNA extraction kit was purchased from Omega Bio Tek (Code No. R6840). Reverse transcription and RT-qPCR kits were purchased from Takara Bio Inc (Code No. RR047A and RR820A), and the experimental steps were strictly carried out according to the instructions. Briefly, the total RNA of the sample was extracted and reverse transcribed into cDNA, following which the cDNA was diluted. RT-qPCR amplification was conducted to verify the efficiency of primer amplification, which was used as a reference gene to normalize expression levels between samples. Primers were designed using Primer 5. The 2-ΔΔCt method was used to characterize the differential expression multiple of genes. The Ct value was defined as the number of cycles required for the fluorescence signal of the amplification product to reach a set threshold during the PCR process.
MDA levels were determined using the thiobarbituric acid method11. GSH-Px was measured using the 5,5’-dithiobis-(2-nitrobenzoic acid) method (DTNB); the concentration of GSH in the reaction system decreasing by 1 μmol/L was an enzyme activity unit (AU)12. The ROS level was measured using the 2’,7'-dichlorodihydrofluorescein diacetate method, and fluorescence intensity was read by a microplate reader for relative comparison (Arbitrary Unit, AU)13. A-CoA was measured using a double-antibody sandwich enzyme-linked immunosorbent assay14. These tests were performed according to the manufacturer’s instructions. Biomass was estimated as the ratio of cell dry matter weight to fermentation broth weight.
The DHA content in the fermentation broth was determined via gas chromatography using the detection principle described by Alinafiah et al.15. Sample pretreatment: (1) lipid extraction: a quantitative amount of Schizochytrium spp. (dry matter) was weighed and added to the tubes. Anhydrous ethanol (10 mL), anhydrous ether (25 mL), and petroleum ether (25 mL) were added, shaken, mixed well, and transferred to a ground flat-bottom flask; the process was repeated thrice. Next, the solvent was rotated and evaporated at 50 °C to near dryness; (2) Saponification and esterification: the concentrate obtained from (1) was added to a methanol solution of 0.5 mol/L potassium hydroxide, refluxed on a water bath at 70 °C for 10 min, and treated with 5 mL of a 13% mass fraction boron trifluoride methanol solution. After refluxing for 10 min, the mixture was transferred to a centrifuge tube. Next, 10 mL of n-hexane was added as the internal standard, the mixture was centrifuged (4400 × g), and the supernatant was passed through anhydrous sodium sulfate for testing.
The Se content of the samples was determined via hydride generation atomic fluorescence spectrometry. Sample processing and operating conditions described by Sun and Feng16 were followed. Briefly, (1) the pretreatment for inorganic Se detection: first, 0.1 g of the obtained Schizochytrium sp. dry powder was accurately weighed and treated with 10 mL of 3 mol/L hydrochloric acid. After disrupting the ultrasonic walls for 5 min, the mixture was placed in a boiling water bath for 10 min, centrifuged (17,700 × g) for 10 min, and the supernatant was collected; these steps were repeated twice, and the supernatants were merged. (2) The pretreatment for total Se detection: the precipitate obtained from (1) was treated with 20 mL nitric acid/perchloric acid mixture (4/1, v/v). After mixing the treated mixture with the supernatant, it was slowly heated on an electric heating plate until it was nearly dry and dissolved in 5 mL of HCl at a concentration of 6 mol/L for testing. (3) The samples obtained from steps (1) and (2) were detected using atomic fluorescence spectrophotometer to determine the content of inorganic Se and total Se, respectively. The organic Se content was calculated by subtracting the inorganic Se content from the total Se content.
The obtained data were preliminarily sorted and analyzed using Excel. Statistical analyses were performed using SPSS 22.0. An ANOVA was used for comparing statistical differences, and Tukey’s method was used for multiple comparisons. The results were expressed as mean ± standard deviation, and P < 0.05 was considered a significant difference.
Changes in the biomass and DHA content of Schizochytrium sp. under different Na2SeO3 supplementation doses showed consistency. Compared to group C, groups T1 and T2 showed an increase in biomass (group T1 and T2 significantly increased by 14.34% and 17.28%, respectively; P = 0.004 and P = 0.001) and DHA content (group T2 significantly increased 40.74%, P = 0.001), while groups T3 and T4 did not show a significant promoting effect, but an inhibition trend instead (Fig. 1). These findings indicated that the biomass and DHA content of Schizochytrium sp. were promoted at appropriate concentrations of Na2SeO3 but inhibited by higher or increased Na2SeO3 concentrations.
Effect of supplying Na2SeO3 on fermentation results. Different lowercase letters “abc” represent significant differences between different treatments of same data (P < 0.05).
The total Se and organic Se in Schizochytrium sp. Increased significantly (P < 0.05) due to supplementation with Na2SeO3 in a dose-dependent manner (Fig. 2). However, there were no statistically significant differences between the Se conversion rates.
Effect of supplying Na2SeO3 on Se content. Different lowercase letters “abc” represent significant differences between treatments for the same data (P < 0.05).
where added Se is calculated based on mass fraction of Se in Na2SeO3;
Se was not detected in control group C, so it is not shown here.
The ratio of organic Se in Schizochytrium sp. also showed a downward trend, wherein that of group T1 was significantly higher than that of the other groups; meanwhile, that of the T4 group was significantly lower than those of the T2 and T3 groups (P = 0.001 and P = 0.021). The results showed that, although the content of total Se and Organic Se increased with increasing Na2SeO3 during the fermentation process of Schizochytrium sp., the utilization efficiency of organic Se decreased. The organic Se conversion rate in group T1 was 100%. There may be two possible reasons for this result: (1) due to the low content of Na2SeO3 (0.1 mg/L) supplementation in group T1, the level of inorganic selenium was lower than the detection limit. Therefore, the organic selenium content was calculated to be 100%. (2) The utilization rate by Schizochytrium sp. may be too high for the small amount of Na2SeO3 involved, resulting in almost all of it being converted to organic Se.
The biochemical test results for each group showed a certain regularity (Fig. 3). Although group T2 was slightly higher than the other groups in A-COA, there was no significant difference. As the concentration of Na2SeO3 increased, the MDA levels decreased in a dose-dependent manner, and the MDA levels in all treatment groups were significantly lower than those in group C (the significance of groups T1, T2, T3, and T4 were P = 0.001, P < 0.001, P < 0.001 and P = 0.001). As the Na2SeO3 concentration increased, GSH-Px activity first increased and then decreased. GSH-Px activity in the T1 and T2 groups was significantly higher (P = 0.007 and P = 0.001) than in group C. However, the level of ROS in group T2 was significantly lower than that in T1 (P = 0.008), and the level in group T1 was significantly lower (P < 0.05) than that in group C (P = 0.010).
Comparison of A-COA, MDA, GSH-Px, and ROS levels in each group. Different lowercase letters “abc” represent significant differences between different treatments of same data (P < 0.05).
The raw sequencing data contained low-quality reads, joint contamination, and a high content of unknown bases. To ensure the reliability of the results, these reads were removed before data analysis. See Table S1 for the quality index of filtered reads. The clean reads ratio of each group was greater than 90%, guaranteeing its reliability. Trinity was used to assemble clean reads and cluster the transcripts to obtain UniGene. TIGR Gene Indices clustering tools were used to cluster the UniGene of each sample again to get the final UniGene, named “all UniGene,” for subsequent analysis (Fig. S1).
To obtain more comprehensive information on gene function, we annotated the longest transcript with reference to seven databases (NR, NT, Pfam, GO, KOG, SwissProt, and KEGG). Five databases (NR, NT, KOG, KEGG, and GO) with similar research purposes and high annotation success rates were selected to draw a Venn diagram (Fig. S2). Although 3474 genes could be correctly annotated in the five databases, many could not. This low annotation was attributed to two factors: (i) some transcriptome data consisted of noncoding sequences, and (ii) Schizochytrium spp. are not well studied, with only a few notes regarding this species and related species being found in existing databases.
Deseq2 was used to analyze and detect the DEGs between samples according to the gene expression level of each sample. The screening threshold for differential genes was Padj < 0.05 and | log2foldchange |> 1. If the log2 fold-change of the gene was > 0, that DEG was considered upregulated; otherwise, the DEG was considered downregulated.
Group T2, the key research group here, showed a large increase in DHA and biomass compared with group C. In contrast, group T4 showed a large degree of inhibition compared with group C, attributed to the inhibition effect exerted by a high dose of Na2SeO3, which was used in a negative regulation study. The pathway research of this study is based on the KEGG database17,18,19. The differential pathway classifications of the DEGs between groups C, T2, and T4 in the KEGG database are shown (Fig. 4a). The supplementation of Schizochytrium sp. with Na2SeO3 may affect transcriptional expression in different pathways, and the number of DEGs in group T4 group was much higher than that in group T2. These results indicated that moderate concentrations of Na2SeO3 (T2) increased the biomass and DHA content by affecting the DEGs of Schizochytrium sp. In contrast, excessive Na2SeO3 (T4) concentrations produced more complex changes in transcriptional expression, resulting in inhibitory effects.
Classification of DEGs in KEGG (a) and M-versus-A plot of DEGs in T2 and T4 (b and c). In M-versus-A plot, X-axis represents A (average log2 gene expression), and Y-axis represents M (log2 fold change). Color red represents upregulated DEGs, color blue represents downregulated DEGs, and color gray represents non-DEGs.
According to the DEGs M-versus-A plots of the T2 and T4 groups (Fig. 4b,c), the T4 group exhibited more DEGs than the T2 group, with a significantly higher proportion of downregulated genes. Based on the research direction, gene expression characteristics, and results, eight representative genes highly likely to be associated with the results were selected for verification via real-time q-PCR (Table 1).
To verify the reliability of transcriptome results, the relative expression difference multiple of the selected genes was calculated using Group C as the reference; the RNA-seq was calculated via log2Ratio, and the RT-qPCR was calculated via −ΔΔCt. The gene expression trends of RNA-seq and RT-qPCR were similar, indicating that the transcriptome sequencing results were reliable (Fig. 5).
Comparison of RNA-seq and RT-qPCR results of gene mRNA expression level.
The GK pathway plays an important role in glycerol metabolism. The results showed that the GK expression in group T2 was significantly higher than in group C (Fig. 5f). Schizochytrium sp. utilizes glucose to generate glycerol via glycolysis and glycerol 3-phosphate (G3P) via GK to generate dihydroxyacetone phosphate (DHAP)20. DHAP and glyceraldehyde triphosphate oscillate between each other through the action of triose phosphate isomerase, although they are restricted to some extent by the dose concentration21. Glyceraldehyde triphosphate generates pyruvate via glyceraldehyde kinase and 3-phosphate glycerate mutase22. In organisms, pyruvate is converted into A-COA, NADH, and CO2 via the pyruvate dehydrogenase complex23. In the PKS pathway, A-COA is a substrate necessary for DHA synthesis24. Therefore, supplementing a Schizochytrium sp. culture medium with Na2SeO3 can promote the synthesis of A-COA via the GK pathway, thereby promoting the synthesis of DHA via the PKS pathway25. However, although the A-COA content in the T2 group was slightly higher than that in the other groups, the difference was insignificant (Fig. 3a). This may be due to the participation of A-COA in many biochemical reactions simultaneously, such as the tricarboxylic acid cycle, causing its content to undergo complex dynamic changes26, thereby preventing the difference in COA content from being detected by this test.
Additionally, a metabolite of the GK pathway, G3P, may synergize with the glycerol promoter. An experiment exploring halophiles found that glycerol kinase activity is regulated by the concentration of environmental glycerol metabolites27. This indicated that an increase in glycerol concentration may enhance the expression of glycerol kinase and that the glycerol promoter is linked to an increase in the efficiency of glycerol phosphorylation28. The DHA present in Schizochytrium spp. has a triglyceride structure, and DHAP and G3P, as important precursors for glycerol synthesis, directly affect the synthesis of glycerol esters29,30. Therefore, promoting the GK pathway plays an important role in forming the triglyceride DHA here.
Selenium is a trace element that is essential for the growth and metabolism of most microorganisms. Usually, microorganisms cannot process Na2SeO3 directly and thus convert Na2SeO3 into selenophosphate via selenophosphate synthetase SelD/SPS2 to initiate antioxidant functions31. Increased cysK expression, thus, increased L-cysteine production (Fig. 5d). The results of the DEG analysis showed that peroxiredoxin (GPX) was significantly expressed in the T2, T3, and T4 groups (Fig. 5c). This suggests that in the presence of Na2SeO3, Schizochytrium spp. utilize cysteine, glutamic acid, and glycine to produce glutathione (GSH). GSH then forms GSH-Px with the aid of glutathione reductase (GR)32. The addition of an appropriate amount of Na2SeO3 to the fermentation broth increased GSH-Px activity and significantly reduced (P < 0.05) ROS and MDA levels (Fig. 3b–d). Large amounts of ROS, which destroy cell integrity via the removal of electrons, are produced during the metabolism and proliferation of Schizochytrium spp.33. Additionally, ROS oxidize DHA synthesized by Schizochytrium sp. to MDA, reducing the production of DHA and attenuating growth34. This study proved that the supplementation of Schizochytrium sp. with an appropriate amount of Na2SeO3 promotes the expression of cysK, increases the activity of GSH-Px, and reduces the ROS and MDA levels, which is closely associated with increases in Schizochytrium sp. biomass and DHA content.
PKS is the main pathway associated with DHA synthesis in Schizochytrium spp.6. The route of this pathway is composed of β-ketoacyl synthetase, β-ketoyl-ACP reductase, enoyl reductase, dehydratase/isomerase, acyltransferase, and acyl carrier protein, which enable its completion. In the PKS pathway, A-COA and malonyl-CoA are used as substrates and undergo condensation, reduction, dehydration, and isomerization cycles, ultimately forming DHA35. The type II PKS pathway consists of a constant minimum PKS core, including a malonyl acyltransferase, an acyl carrier protein (ACP), and an α/β Isodimer ketone synthase (KS α-KS β), with the smallest PKS core dominating the main functions of this approach36,37, wherein, KS α plays an assembly role38,39. Bräuer et al.40 studied the type II PKS pathway using X-ray crystallography. They confirmed the presence of a flexible cysteine binding site (cys176) in KSα, which enables the flexible cysteamine group to transfer the malonyl building block from 4'-phosphopantetheine to the ketosynthases in cys176. Here, the cysteine synthase expression increased, which increased the synthesis of L-cysteine. Thus, we deduced that using cysteine by KSα in the PKS pathway improved and promoted DHA synthesis.
When the Na2SeO3 supplement reached 1 mg/L, the biomass and DHA content of Schizochytrium sp. began to decline, and the growth itself was inhibited when Na2SeO3 reached 5 mg/L (Fig. 1b). As Na2SeO3 continued to be added, the utilization rate of Se by Schizochytrium sp. showed a downward trend (Fig. 2b). This indicated that a high dose of Na2SeO3 could not be fully utilized by Schizochytrium sp. Nine significantly downregulated DEGs that may inhibit the growth and metabolism of Schizochytrium spp. were identified via screening (Table 2). Oxalate-CoA ligase participates in the tricarboxylic acid cycle41. Acyl-CoA oxidase is closely related to forming substrates for fatty acid synthesis42. Furthermore, 2,4-dienoyl-CoA reductase participates in polyunsaturated fatty acid β oxidation43. Acetyl-CoA synthetase is closely associated with the production of cellular triglycerides44. Acyl-CoA dehydrogenase plays an important role in mitochondrial metabolism45. The fermentation broth of Schizochytrium sp. is a high-salt environment, and choline dehydrogenase plays an important role in salt tolerance46. Signaling mucin MSB2 promotes the filamentous growth of yeast cells, indicating its association with cell division47. Glutamate dehydrogenase is a common catalytic enzyme in glutamic acid metabolism48. Aldehyde dehydrogenase reduces the toxic effects of ethanol metabolism by producing acetaldehyde49. The expression of these key enzymes plays a role in the growth and metabolism of Schizochytrium spp. Thus, a decreased expression of these enzymes may account for the lowering of its biomass and DHA content. However, judging by the number of DEGs, the reasons for the inhibitory effects exerted by an excess of Na2SeO3 on Schizochytrium spp. are complicated, and further investigations were warranted.
Interestingly, although the expression level of GPX significantly increased in groups T3 and T4 (Fig. 5c), GSH-Px activity was significantly lower than that in group T2 (P < 0.05) (Fig. 3c). In contrast, there was no significant difference between the levels of MDA and ROS in these groups (Fig. 3b and d). When microorganisms are exposed to selenite (SeO32−) toxicity, mercaptan can react with SeO32− and achieve detoxification50. GSH is a mercaptan commonly found in Schizochytrium spp. GSH reacts with SeO32− to generate selenium-glutathione (GS-Se-SG), which is further reduced to GS-Se- via the action of GR and thioredoxin reductase (TR), and hydrolyzed to GSH and se0, during which process many ROS are generated51. This indicates that with an excess of Na2SeO3, GSH will consume GR to detoxify SeO32− by acting as a “catalyst” by changing se-4 to red se0, which prevents GSH from converting to GSH-Px, and limits its ability to remove ROS and MDA.
It was observed that the fermentation broth of group T4 was redder than that of group C. We confirmed that there was a large amount of se0 in the fermentation broth of the T4 Schizochytrium sp. The ratio of organic Se in group T4 decreased significantly (P < 0.05), indicating that the ratio of inorganic Se (se0) in Schizochytrium sp. had increased; this finding was consistent with the above inference (Fig. 2). During this process, selenium was mostly present in the form of selenoamino acids, which explained why the organic selenium content still significantly increased even though high doses of sodium selenite had inhibitory effects (Fig. 1).
The mechanism of the effect of Na2SeO3 on Schizochytrium sp. is shown in Fig. 6. Adding an appropriate amount of Na2SeO3 (0.5 mg/L) during the fermentation process of Schizochytrium sp. promotes the growth of its biomass and DHA content. This growth is attributed to promoting GK and cysK pathways and generating GSH-Px. However, any further increase in the Na2SeO3 concentration (1 and 5 mg/L) lowers the biomass and DHA content of Schizochytrium sp. The conversion to organic Se may be achieved using various levels of Na2SeO3 supplementation; however, increasing the amount of Na2SeO3 would decrease the conversion rate of organic Se due to its toxic effects. Thus, this study provides the theoretical basis for a new method to simultaneously enhance the DHA content and produce organic Se, using Schizochytrium sp. In any actual production process, the proportion of Na2SeO3 supplementation may be adjusted based on the economic benefits expected.
Schematic diagram of mechanism of Na2SeO3 effect on Schizochytrium sp.
The datasets generated and/or analysed during the current study are available in the NCBI-SRA database, [https://www.ncbi.nlm.nih.gov/sra/PRJNA1002230].
Drover, J. R. et al. A randomized trial of DHA intake during infancy: school readiness and receptive vocabulary at 2–3.5 years of age. Early Hum. Dev. 88, 885–891 (2012).
Birch, E. E. et al. The DIAMOND (DHA Intake And Measurement Of Neural Development) Study: a double-masked, randomized controlled clinical trial of the maturation of infant visual acuity as a function of the dietary level of docosahexaenoic acid. Am. J. Clin. Nutr. 91(4), 848–859 (2010).
Article CAS PubMed Google Scholar
Xu, J. et al. Docosahexaenoic acid enhances hippocampal insulin sensitivity to promote cognitive function of aged rats on a high-fat diet. J. Adv. Res. 45, 31–42 (2023).
Article CAS PubMed Google Scholar
Grimm, M. O., Zimmer, V. C., Lehmann, J., Grimm, H. S. & Hartmann, T. The impact of cholesterol, DHA, and sphingolipids on Alzheimer’s disease. BioMed Res. Int. 2013, 814390; https://doi.org/10.1155/2013/814390 (2013).
Watanabe, Y. & Tatsuno, I. Omega-3 polyunsaturated fatty acids focusing on eicosapentaenoic acid and docosahexaenoic acid in the prevention of cardiovascular diseases: a review of the state-of-the-art. Expert Rev. Clin. Pharmacol. 14, 79–93 (2021).
Article CAS PubMed Google Scholar
Shi, Y. et al. Function of ORFC of the polyketide synthase gene cluster on fatty acid accumulation in Schizochytrium limacinum SR21. Biotechnol. Biofuels. 14, 163 (2021).
Article CAS PubMed PubMed Central Google Scholar
Ferrari, L. et al. Advances in selenium supplementation: From selenium-enriched yeast to potential selenium-enriched insects, and selenium nanoparticles. Anim Nutr. 14, 193–203 (2023).
Article CAS PubMed PubMed Central Google Scholar
Esmaeili, S. & Khosravi-Darani, K. Selenium-enriched yeast: as selenium source for nutritional purpose. Curr. Nutr. Food Sci. 10, 49–56 (2014).
Nancharaiah, Y. V. & Lens, P. N. Ecology and biotechnology of selenium-respiring bacteria. Microbiol. Mol. Biol. Rev. 79, 61–80 (2015).
Article CAS PubMed PubMed Central Google Scholar
Wang. Y. et al. Selenite Reduction by Proteus sp. YS02: New Insights Revealed by Comparative Transcriptomics and Antibacterial Effectiveness of the Biogenic Se0 Nanoparticles. Front Microbiol. 13, 845321; https://doi.org/10.3389/fmicb.2022.845321 (2022).
Liao, S. et al. Transcriptome analysis of protocatechualdehyde against listeria monocytogenes and its effect on chicken quality characteristics. Foods. 12, 2625. https://doi.org/10.3390/foods12132625 (2023).
Article CAS PubMed PubMed Central Google Scholar
Bu, Y. et al. Silver-nanoparticle-embedded porous silicon disks enabled SERS signal amplification for selective glutathione detection. ACS Appl. Nano Mater. 1, 410–417 (2018).
Article CAS PubMed Google Scholar
Li, X., Hu, H. Y. & Zhang, Y. P. Growth and lipid accumulation properties of a freshwater microalga Scenedesmus sp. under different cultivation temperature. Bioresour. Technol. 102, 3098–3102 (2011).
Li, L., Peng, M., Ge, C., Yu, L. & Ma, H. (-)-Hydroxycitric acid reduced lipid droplets accumulation via decreasing acetyl-coa supply and accelerating energy metabolism in cultured primary chicken hepatocytes. Cell Physiol. Biochem. 43, 812–831 (2017).
Article CAS PubMed Google Scholar
Alinafiah, S. M., Azlan, A., Ismail, A. & Mahmud Ab Rashid, N. Method development and validation for omega-3 fatty acids (DHA and EPA) in fish using gas chromatography with flame ionization detection (GC-FID). Molecules. 26, 6592 (2021).
Sun, H. & Feng, B. Speciation of organic and inorganic selenium in selenium-enriched eggs by hydride generation atomic fluorescence spectrometry. Food Anal. Methods. 4, 240–244 (2011).
Ogata, H. et al. KEGG: kyoto encyclopedia of genes and genomes. Nucleic Acids Res. 27, 29–34 (2000).
Kanehisa, M. Toward understanding the origin and evolution of cellular organisms. Protein Sci. 28, 1947–1951 (2019).
Article CAS PubMed PubMed Central Google Scholar
Kanehisa, M. et al. KEGG for taxonomy-based analysis of pathways and genomes. Nucleic Acids Res. 51, D587–D592 (2023).
Article CAS PubMed Google Scholar
Zhang, Y. et al. Simultaneous production of 1,3-dihydroxyacetone and xylitol from glycerol and xylose using a nanoparticle-supported multi-enzyme system with in situ cofactor regeneration. Bioresour. Technol. 102, 1837–1843 (2011).
Article CAS PubMed Google Scholar
Wierenga , RK , Kapetaniou , EG & Venkatesan , R. Triosephosphate isomerase: A highly evolved biocatalyst .Cell Mol.Life Sci.67, 3961–3982 (2010).
Article CAS PubMed Google Scholar
Hitosugi, T. et al. Phosphoglycerate mutase 1 coordinates glycolysis and biosynthesis to promote tumor growth. Cancer Cell 22, 585–600 (2012).
Article CAS PubMed PubMed Central Google Scholar
Igamberdiev, A. U., Lernmark, U. & Gardeström, P. Activity of the mitochondrial pyruvate dehydrogenase complex in plants is stimulated in the presence of malate. Mitochondrion 19, 184–190 (2014).
Article CAS PubMed Google Scholar
Pietrocola , F. , Galluzzi , L. , Bravo-San Pedro , JM , Madeo , F. & Kroemer , G. Acetyl coenzyme A: A central metabolite and second messenger .Cell Metab.Rev. 21, 805–821 (2015).
Xie, Y. & Wang, G. Mechanisms of fatty acid synthesis in marine fungus-like protists. Appl. Microbiol. Biotechnol. 99, 8363–8375 (2015).
Article CAS PubMed Google Scholar
Zhang, S. & Bryant, D. A. The tricarboxylic acid cycle in cyanobacteria. Science 334, 1551–1553 (2011).
Article ADS CAS PubMed Google Scholar
Ouellette. M., Makkay, A. M., Papke, R. T. Dihydroxyacetone metabolism in Haloferax volcanii. Front Microbiol. 4, 376; https://doi.org/10.3389/fmicb.2013.00376 (2013).
Lee, Y. J., Jeschke, G. R., Roelants, F. M., Thorner, J. & Turk, B. E. Reciprocal phosphorylation of yeast glycerol-3-phosphate dehydrogenases in adaptation to distinct types of stress. Mol. Cell Biol. 32, 4705–4717 (2012).
Article CAS PubMed PubMed Central Google Scholar
Hao, G., Chen, H., Gu, Z., Zhang, H., Chen, W., & Chen, Y. Q.. Metabolic engineering of Mortierella alpina for arachidonic acid production with glycerol as carbon source. Microb Cell Fact. 14, 205; https://doi.org/10.1186/s12934-015-0392-4 (2015).
Ma, W., et al. Efficient co-production of EPA and DHA by Schizochytrium sp. via regulation of the polyketide synthase pathway. Commun Biol. 5, 1356; https://doi.org/10.1038/s42003-022-04334-4. (2022).
Manta, B., Makarova, N. E. & Mariotti, M. The selenophosphate synthetase family: a review. Free Radic. Biol. Med. 192, 63–76 (2022).
Article CAS PubMed Google Scholar
Franklin, C. C. et al. Structure, function, and post-translational regulation of the catalytic and modifier subunits of glutamate cysteine ligase. Mol. Aspects Med. 30, 86–98 (2009).
Article CAS PubMed Google Scholar
Tosi, S. et al. Antioxidant enzyme activity of filamentous fungi isolated from Livingston Island. Maritime Antarctica. Polar. Biol. 33, 1227–1237 (2010).
Eze, J. I., Anene, B. M. & Chukwu, C. C. Determination of serum and organ malondialdehyde (MDA) concentration, a lipid peroxidation index, in Trypanosoma brucei-infected rats. Comp. Clin. Pathol. 18, 209 (2009).
Meesapyodsuk, D. & Qiu, X. Biosynthetic mechanism of very long chain polyunsaturated fatty acids in Thraustochytrium sp. 26185. J. Lipid Res. 57, 1854–1864 (2016).
Chen, A., Re, R. N. & Burkart, M. D. Type II fatty acid and polyketide synthases: deciphering protein-protein and protein-substrate interactions. Nat. Prod. Rep. 35, 1029–1045 (2018).
Article CAS PubMed PubMed Central Google Scholar
Wesener, S. R., Potharla, V. Y. & Cheng, Y. Q. Reconstitution of the FK228 biosynthetic pathway reveals cross talk between modular polyketide synthases and fatty acid synthase. Appl Environ Microbiol. 77, 1501–1507 (2011).
Article ADS CAS PubMed Google Scholar
Mindrebo, J. T. et al. Structure and mechanistic analyses of the gating mechanism of elongating ketosynthases. ACS Catal. 11, 6787–6799 (2021).
Article CAS PubMed PubMed Central Google Scholar
Chen, A., Re, R. N. & Burkart, M. D. Type II fatty acid and polyketide synthases: Deciphering protein–protein and protein–substrate interactions. Nat. Prod. Rep. 35, 1029–1045 (2018).
Article CAS PubMed PubMed Central Google Scholar
Bräuer, A. et al. Structural snapshots of the minimal PKS system responsible for octaketide biosynthesis. Nat. Chem. 12, 755–763 (2020).
Xian, P. et al. Wild soybean oxalyl-CoA synthetase degrades oxalate and affects the tolerance to cadmium and aluminum stresses. Int. J. Mol. Sci. 21, 8869 (2020).
Article CAS PubMed PubMed Central Google Scholar
Fabiszewska, A., Misiukiewicz-Stępień, P., Paplińska-Goryca, M., Zeniuk, B. & Białecka-Florjańczyk, E. An Insight into Storage Lipid Synthesis by Yarrowia lipolytica Yeast Relating to Lipid and Sugar Substrates Metabolism.Biomolecules.9, 685;https://doi.org/10.3390/biom9110685 (2019).
Semini, G. et al. Leishmania encodes a bacterium-like 2,4-dienoyl-coenzyme A reductase that is required for fatty acid β-Oxidation and intracellular parasite survival. mBio. 11, e01057–20 (2020).
Avidan, O. & Pick, U. Acetyl-CoA synthetase is activated as part of the PDH-bypass in the oleaginous green alga Chlorella desiccata. J. Exp. Bot. 66, 7287–7298 (2015).
Article CAS PubMed PubMed Central Google Scholar
Nouws, J. et al. Acyl-CoA dehydrogenase 9 is required for the biogenesis of oxidative phosphorylation complex I (J). Cell Metab. 12, 283–294 (2010).
Article CAS PubMed Google Scholar
Giri, J. Glycinebetaine and abiotic stress tolerance in plants. Plant Signal Behav. 6, 1746–1751 (2011).
Article CAS PubMed PubMed Central Google Scholar
Lanver, D., Mendoza-Mendoza, A., Brachmann, A. & Kahmann, R. Sho1 and Msb2-related proteins regulate appressorium development in the smut fungus Ustilago maydis. Plant Cell 22, 2085–2101 (2010).
Article CAS PubMed PubMed Central Google Scholar
Jayaraman, V. et al. A counter-enzyme complex regulates glutamate metabolism in Bacillus subtilis. Nat Chem Biol. 18, 161–170 (2022).
Article CAS PubMed Google Scholar
Stagos, D. et al. Aldehyde dehydrogenase 1B1: molecular cloning and characterization of a novel mitochondrial acetaldehyde-metabolizing enzyme. Drug Metab. Dispos. 38, 1679–1687 (2010).
Article CAS PubMed PubMed Central Google Scholar
Kharma, A. et al. Inorganic Polysulfides and Related Reactive Sulfur–Selenium Species from the Perspective of Chemistry. Molecules. 24, 1359; https://doi.org/10.3390/molecules24071359 (2019).
Miller, C. G. & Schmidt, E. E. Disulfide reductase systems in liver. Br. J. Pharmacol. 1, 532–543 (2019).
This work was financially supported by the National Key Research and Development Program of China (No. 2017YFD0501500), the Hunan Graduate Research Innovation Project (No. QL20210159), Hunan Canzoho Biological Technology Co., Ltd., and Changsha Selenized Schizochytrium sp. Peptide Fermentation Technology Innovation Center. We would like to thank Editage (www.editage.cn) for the English language editing.
Hunan Agricultural University Veterinary Faculty, No.1 Nongda Road, Furong District, Changsha City, 410000, Hunan, China
Yunqiang Zhang, Zikui Liu, Gang Xiao, Jiawei Shi, Ning Xiao & Zhiliang Sun
Hunan Canzoho Biological Technology Co., Ltd., 321 Kangning Road, Changsha City, 410000, Hunan, China
Yunqiang Zhang, Zikui Liu, Jiawei Shi & Baili Liu
You can also search for this author in PubMed Google Scholar
You can also search for this author in PubMed Google Scholar
You can also search for this author in PubMed Google Scholar
You can also search for this author in PubMed Google Scholar
You can also search for this author in PubMed Google Scholar
You can also search for this author in PubMed Google Scholar
You can also search for this author in PubMed Google Scholar
Y. Z. and Z. S. wrote the main manuscript text and Y. Z. prepared figures 1-6. G. X., N. X., J. S. and B. L. conducted data organization. All authors reviewed the manuscript.
The authors declare no competing interests.
Springer Nature remains neutral with regard to jurisdictional claims in published maps and institutional affiliations.
Open Access This article is licensed under a Creative Commons Attribution 4.0 International License, which permits use, sharing, adaptation, distribution and reproduction in any medium or format, as long as you give appropriate credit to the original author(s) and the source, provide a link to the Creative Commons licence, and indicate if changes were made. The images or other third party material in this article are included in the article's Creative Commons licence, unless indicated otherwise in a credit line to the material. If material is not included in the article's Creative Commons licence and your intended use is not permitted by statutory regulation or exceeds the permitted use, you will need to obtain permission directly from the copyright holder. To view a copy of this licence, visit http://creativecommons.org/licenses/by/4.0/.
Zhang, Y., Liu, Z., Xiao, G. et al. Simultaneous DHA and organic selenium production by Schizochytrium sp.: a theoretical basis. Sci Rep 13, 15607 (2023). https://doi.org/10.1038/s41598-023-42900-w
DOI: https://doi.org/10.1038/s41598-023-42900-w
Anyone you share the following link with will be able to read this content:
Sorry, a shareable link is not currently available for this article.
Provided by the Springer Nature SharedIt content-sharing initiative
By submitting a comment you agree to abide by our Terms and Community Guidelines. If you find something abusive or that does not comply with our terms or guidelines please flag it as inappropriate.
Scientific Reports (Sci Rep) ISSN 2045-2322 (online)

Chlorella Algae Sign up for the Nature Briefing newsletter — what matters in science, free to your inbox daily.